- The two major types of bonding in chemical compounds
- To explain why both types exist and when they are likely to form.
- The varying properties of ionic and covalent bonding.
- How to predict the formula of ionic and covalent compounds.
Notes:
- In Introduction to bonding, we saw the two broad categories of bonding: intermolecular forces (the forces acting between molecules) and intramolecular forces (the bonds within molecules). Molecules of a substance are held together by intramolecular forces - chemical bonds between the atoms that the substance is made of.
- For example, CO2 is carbon dioxide and it is carbon dioxide because it is made of one carbon atom bonding to two oxygen atoms, sitting between them in the middle of the molecule. If this arrangement changes, CO2 changes into something else.
- One major type of chemical bond is the ionic bond:
- Ionic bonding is a bonding attraction caused by oppositely charged ions interacting with each other. It occurs between metal and non-metal atoms.
- To obtain a full outer shell, metal atoms can donate their outer shell electrons to non-metal atoms. Having lost electrons, the metal atom has become a positive ion and the non-metal atom that gained them is now a negative ion.
- The transfer of electrons is driven by the stability of having a full outer shell and the difference in electronegativity. The non-metal atom will have a much higher electronegativity value than the metal atom.
- The force of attraction in an ionic bond is the oppositely charged ions interacting with each other. Electrostatic principles apply: a 2+ metal ion with a 2- nonmetal ion is a stronger ionic bond than 1+ with a 1-.
- Examples of simple ionic compounds are sodium chloride, NaCl, or magnesium oxide, MgO.
- Ionic bonds form between elements on opposite sides of the periodic table.
- Periodic trends like atomic radius and the charge of ions will influence ionic bond strength in compounds. These can be explained in terms of electrostatic theory.
- Ions with a smaller atomic radius enable any oppositely charged ions to come into closer contact, which increases ionic bond strength. In other words, opposite charges make a stronger bond when they are closer to each other.
- The larger the charge on an ion, the greater the charge difference will be with the oppositely charged ion, which produces a stronger ionic bond. 2+ will attract 2- stronger than 1+ attracts 1-.
- Another major type of chemical bond is the covalent bond:
- Covalent bonding happens when two atoms share a pair of electrons between them. In any electron pair that make a covalent bond, one electron comes from each atom, hence the name co (cooperating, working together) valent.
- Covalent bonding occurs between non-metal atoms with similar electronegativity. The similar electronegativity is what leads to the electrons being shared, not gained or lost as in an ionic bond. Because covalent bonding involves atoms gaining electrons by sharing them to complete an outer shell, it is very rare to see metal atoms covalently bond.
- The driving force of a covalent bond forming is that both atoms have more completion of their outer shell.
- The force of attraction in a covalent bond is the shared electron pair being attracted to the nuclei of both atoms making the covalent bond.
- Covalent bonding can involve more than one electron pair:
- A covalent bond with one electron pair is a single bond.
- A covalent bond with two electron pairs is a double bond and is almost twice as strong as a single bond.
- A covalent bond with three electron pairs is a triple bond, almost three times as strong as a single bond.
Well see why its almost twice/three times as strong later. - Covalent bonds can vary in length (measured by the distance between the two nuclei), but in general the stronger the bond, the shorter the bond length.
- Covalent bonding requires similar, not equal electronegativity. There can be some variety in this electronegativity gap which creates dipoles, or polar covalent bonds. It is not a black and white issue whether you have a covalent or ionic bond; it is a dial which can be turned from 0 to 100.
- Start by knowing that covalent bonds occur between metal and nonmetal atoms.
- The larger the gap in electronegativity between two atoms, the more polar their covalent bond will be. A polar bond means the two atoms making the bond have opposing partial charges (+ or -). They are not full ions; the atoms are just not sharing the electrons equally because the more electronegative atom has a greater pull on them.
- Because of the opposite partial charges, we have created something of a north/south pole along the bond. We call this a dipole. It is not an ionic bond, but a polar covalent bond. We can also say this covalent bond has slight ionic character.
- This slightly ionic character means that the two atoms are slightly attracted to one anothers opposing charges, like an ionic bond - at least more than if there was zero ionic character!
For this reason, bond polarity tends to make covalent bonds stronger. - When carbon bonds with bromine, there is a small gap in electronegativity where bromine pulls the electrons with greater force than carbon. This means there is only a very slight partial charge on the two atoms and the electrons are almost equally shared. The relatively bulky bromine atom also makes the covalent bond long; the carbon and bromine nuclei are relatively far away from the electron pair they share.
This adds up to a weak C-Br covalent bond with very little polarity. - When carbon bonds with fluorine, there is a very large electronegativity gap. Fluorine pulls the electrons toward itself substantially more than carbon does. This creates large partial + and - charges on carbon and fluorine respectively. The small size of the fluorine atom also means the atoms can be in closer contact and the attractive forces are stronger.
This all adds up to a very strong C-F covalent bond with significant ionic character. - A gap in electronegativity is the easiest way to predict whether ionic or covalent bonding will occur in a chemical substance since it is electronegativity that drives the tendency for electrons to be gained or lost.
- When two atoms with a large gap in electronegativity form a bond, the atom with a higher electronegativity is going to pull a lot more electron density (think of the electrons as clouds).
- You can use the valence of an atom to work out the formula of covalent and ionic compounds:
- For each atom, find the valence (number of unpaired) electrons. This will be the group number up to carbons group. After this, electrons pair up in the outer shell, so it will be 8 minus the group number. This is the valence of the atom. (E.g. N has a valence of 8 - 5 = 3 due to 3 unpaired electrons, H has 1).
- Cross the valence of each atom with the other this is the number of atoms of the element that will combine to form the compound. (N = 1, H = 3 makes NH3, a covalent compound).
- Remember to take the lowest whole-number ratio of atoms e.g. in the C2O4 example, this can be simplified to CO2, or carbon dioxide.
Nuclear charge affects atomic/ionic radius and it helps explain trends seen in isoelectronic ions (ions with the same number of electrons, e.g. N3-, O2-, F-, Na+, Mg2+, Al3+). In a group of these ions, the ion with the greatest nuclear charge has the smallest atomic radius because the increasingly positive nuclear charge attracts the same number of electrons more strongly.
Lets take two examples to show the difference:
When covalent compounds contain polar bonds, the unequal pull of electrons towards some atoms and away from others creates polar molecules. We say that these molecules have permanent dipoles. These are represented with vector arrows that point from the positive to areas of negative charge.
Polarity in molecules has a major effect on intermolecular forces and the solubility of a substance, which we will see in Intermolecular forces and Polarity.
Covalent and ionic bonds occur within molecules, between the atoms and ions that bond to make a substance what it is. They are both strong forces simple ionic compounds often have melting points of several hundred degrees Celsius, as do some large covalent structures.
Example 1:
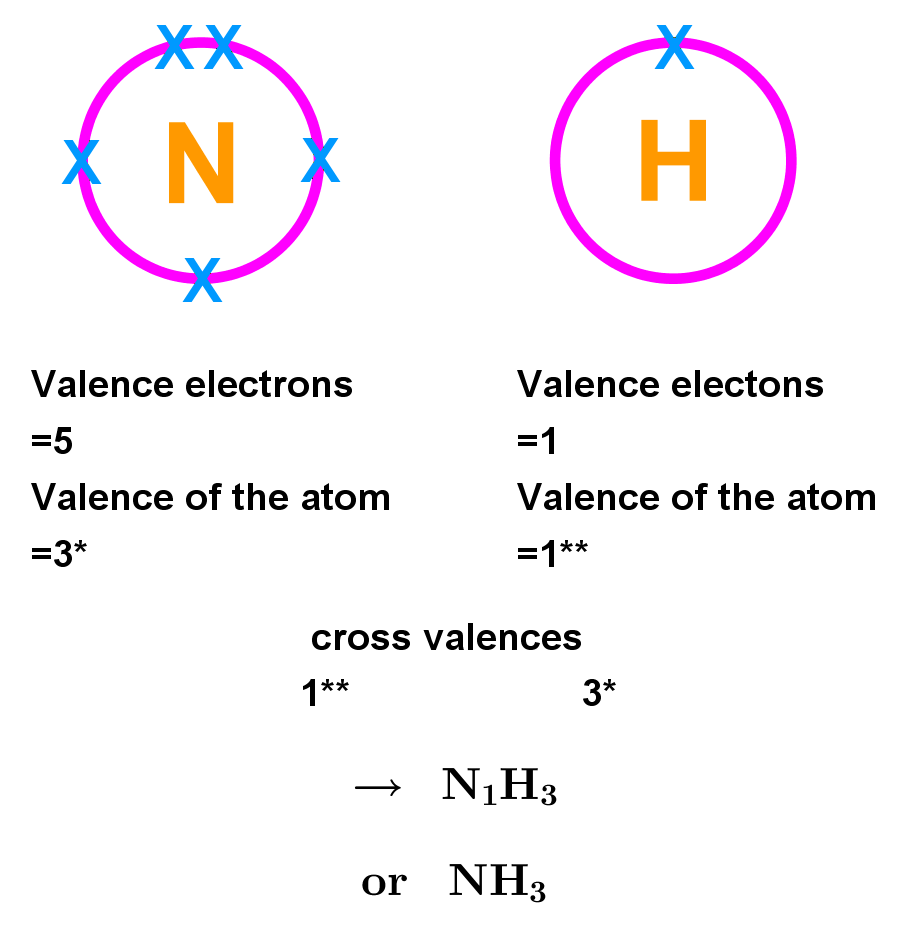
Example 2:
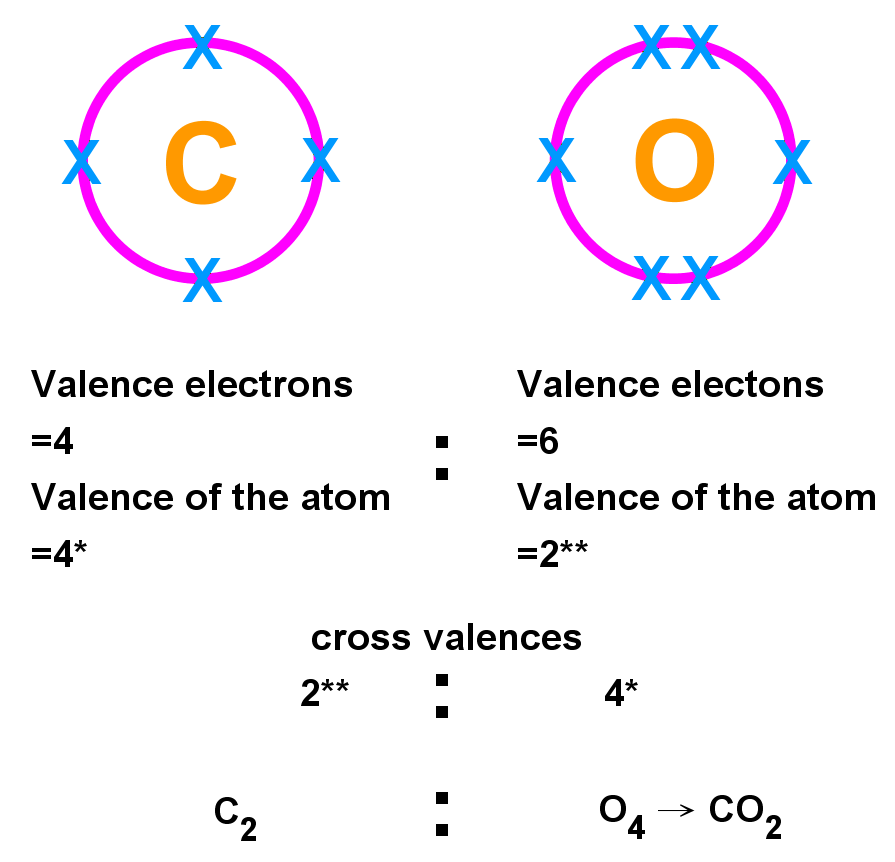
- (IB) Some covalent bonds are made by both electrons being donated by the same atom. This is a coordinate covalent bond. They are normally made by lone pairs on atoms like oxygen or nitrogen donating to H+ or a metal atom.
- For example, in the H2O molecule there are two lone pairs of electrons on oxygen that are not making regular covalent bonds to the hydrogen atoms. These lone pairs can form a covalent bond with a hydrogen ion in solution:
This reaction happens whenever an acid is dissolved in water.