In this lesson, we will learn:
- Magnetic field due to a long straight wire
- Magnetic field midway between two currents
- Forces between two parallel wires
Notes:
An electric current produces a magnetic field
- The magnetic field surrounding the electric current in a long straight wire is such that the field lines are circles with the wire at the center.
- The field strength at a given point would be greater if the current flowing in the wire were greater;
- The filed strength would be less at points farther from the wire ;
,
The value of the constant , which is called the permeability of free space, is = 4 × 10-7
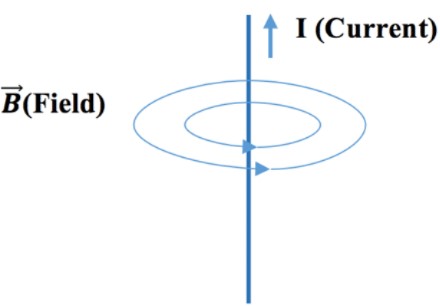
Two parallel wires 10.0cm apart carry currents in opposite directions. Current = 5.0A is out of the page, =7.0 A is into the page. Determine the magnitude and direction of the magnetic field halfway between the two wires.
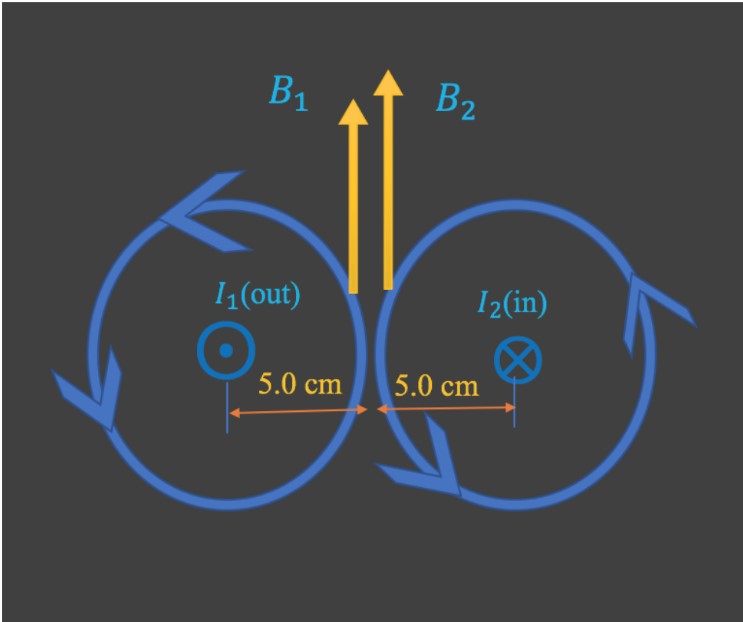
= 2.0 × 10-5
= 2.8 × 10-5
The total filed is up with the magnitude of
- Consider two long parallel wires separated by a distance . They carry currents and , respectively. Each current produces a magnetic field that is felt by the other, so each must exert a force on the other.
- Magnetic field produced by
- The force is exerted by on a length of of wire 2, carrying current , =
- Substitute into formula to get the final equation;
- Parallel currents in the same direction exert an attractive force on each other
- Antiparallel currents (in opposite directions) exert a repulsive force on each other.
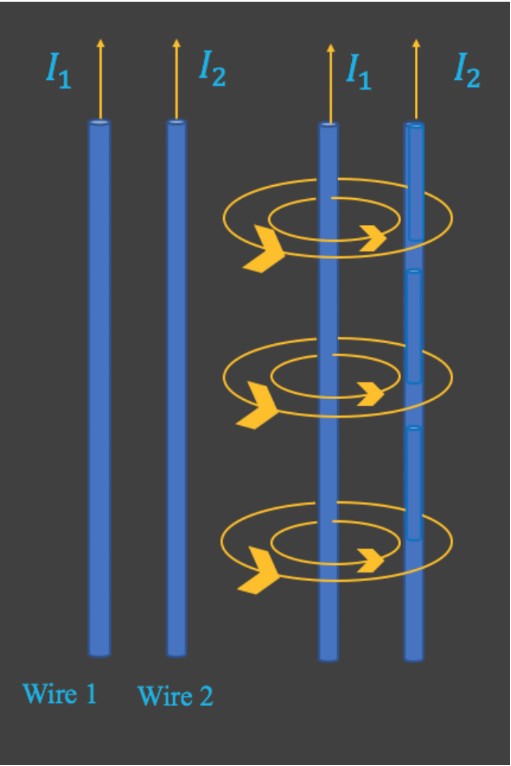
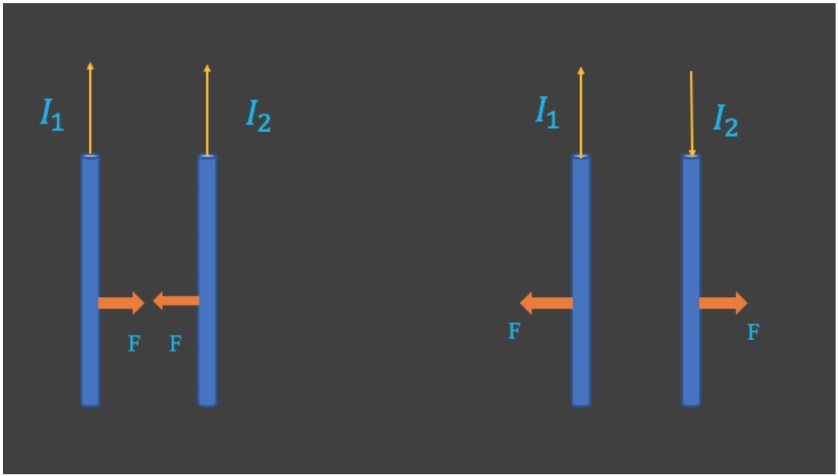