In this lesson, we will learn:
- To understand the experimental evidence that led to our current understanding of electrons and orbitals.
- To recall the shape and nature of early electron orbitals and energy level diagrams.
- To fill electron orbitals for the first two rows of elements correctly according to the Pauli and Aufbau principles.
Notes:
- There are many methods we use to learn about chemical bonding, find out the shape and structure of compounds, but why do atoms even form molecules and compounds in the first place? What causes different molecules to have different shapes?
VSEPR helps us predict molecular shapes. Recall the shapes of some molecules from Molecular Geometry:- BH3 is trigonal planar, but NH3 and PH3, with the same number of atoms, don't form the same shape as BH3.
- CH4 is tetrahedral and isoelectronic molecules (same number of electrons) like NH3 and water H2O still form the same tetrahedral structure without four H atoms, using their lone electron pairs instead.
- Some atoms do not form molecules; for some reason they are stable enough to exist as individual atoms, like helium (He).
Clearly, it is not the number of atoms or number of electrons that determine molecule shape and other properties, it is the different states electrons are in. Today we use atomic orbitals to describe the behaviour of electrons in atoms.
- To understand why we use orbitals to describe electrons it is very helpful to know how science got here. Some extremely important experiments and developments were made in the early 20th century. All the developments below led to Nobel Prizes for the scientists contributions to quantum mechanics:
- In 1900, Max Planck derived Planck’s law, which solved the ultraviolet catastrophe. Classical theory predicted that the emission spectrum of a black body (think of the sun as a rough example) would amount to emitting infinite amounts of UV and other high frequency radiation.
This is obviously not true; humans wouldn’t exist if it was! By assuming energy is transferred and emitted in quantized amounts, Planck’s revision to the obviously flawed theory correctly fitted real observations. - In 1905, Einstein’s experiment, the photoelectric effect, was explained using Planck’s idea of quantization the Planck-Einstein relation, E = hv. Einstein explained that light travels as quantized packets of energy called photons.
Although light was long considered a wave, it also shows particulate properties. - In 1913, Niels Bohr used the quantization of energy to develop his model of the atom. Bohr could explain the atomic emission spectrum (AES) of hydrogen; the electron orbited the nucleus in quantized energy states. If it absorbed energy, an electron would be excited to a higher energy state, before returning to a ground state, emitting a quantized amount of energy (E = hv) in the process. These energy ‘states’ are the electron shells.
- In 1923, the de Broglie hypothesis proposed that like light, all matter including electrons has wave-particle nature. He developed the de Broglie wavelength formula = h/p, which applies to particles like electrons, relating momentum of a particle to wavelength. Later experiments of electrons diffracting through slits (a wave property) confirmed the wave properties of electrons.
- In 1927, Werner Heisenberg developed Heisenberg’s uncertainty principle. A simple consequence of wave-particle duality is that we cannot exactly know the location AND momentum of an electron at the same time.
- Waves have no definitive position; they are continuously spread through space. However, we can identify features of waves like wavelength and frequency, which is related to energy and momentum.
- Particles definitively exist in one position at a given time, but they do not have a wavelength and as such its momentum is unknown.
- In 1926, the Schrodinger equation was developed to describe the wave behaviour of electrons over time using wave functions.
- The most important point from the above is that drawing electrons as particles orbiting around a nucleus is WRONG. Showing electrons in shells ‘orbiting’ around the nucleus suggest they are single point charges (they aren’t) and we know exactly where they are (we don’t).
Electrons are better thought of as ‘clouds’ of density inside orbitals where they are most likely to be found. Orbitals are like electron houses; they aren’t always/definitely there, but they are most likely there (or more likely there than anywhere else). - All orbitals can hold two electrons, no more. This is part of the Pauli principle. This first 1s orbital, then, covers the electrons for hydrogen and helium, which you can show using an energy-level diagram or subshell notation.
- The first, lowest energy orbital is called a 1s orbital.
- 1 refers to an energy level; the lower it is, the more stable it is and the closer to the nucleus it is.
- S refers to shape – think s for sphere .
- After the first two electrons are filled in the 1s orbital, the third and fourth electrons are found in the 2s orbital. This is where the third electron of Li and third/fourth for Be are found.
- Again, s means spherical but 2 shows a higher energy level. This orbital is higher in energy than 1s because the electrons are normally further away from the nucleus.
- The positive region will, for example, overlap with positive parts of other orbitals (constructive interference).
- The positive part will cancel out negative parts of other orbitals (destructive interference).
- The 2nd energy level also has orbitals where the node is not spherical, but in a single plane. These are called p orbitals, and there are three in each energy level - one each for the x, y and z axes they occupy. They are considered “lobe shaped”. See below for images:
- With two electrons each, this gives the electron configurations for the elements boron through neon. See the diagram below for the energy level diagrams:
- The evidence for electron sublevels or subshells largely comes from photoelectron spectroscopy (PES), a development from the photoelectric effect. This is covered in our lesson Photoelectron spectroscopy.
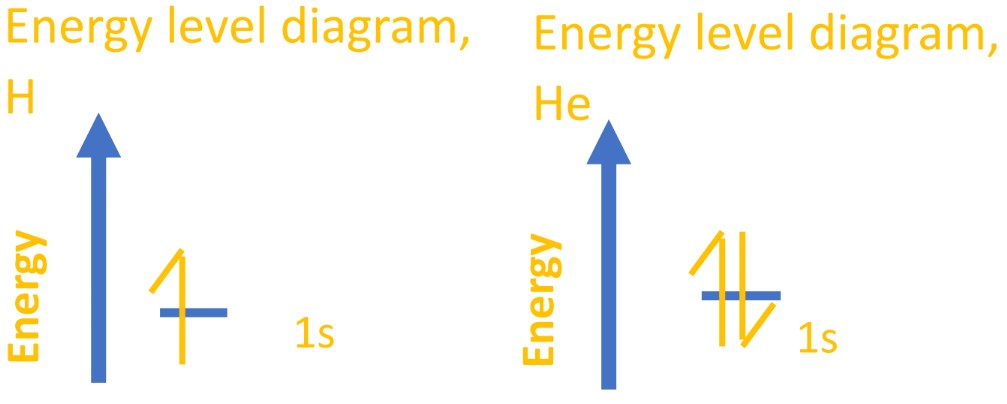
Because this zero node separates the orbital into two halves, it is convention to draw these halves as opposing regions (red/blue, +/-, shaded/unshaded). This is how we try and show the part wave nature of the electrons in orbitals:
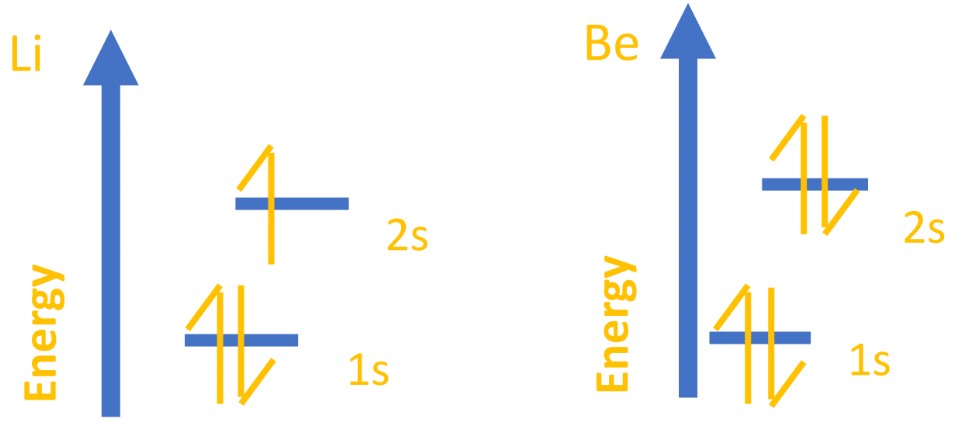
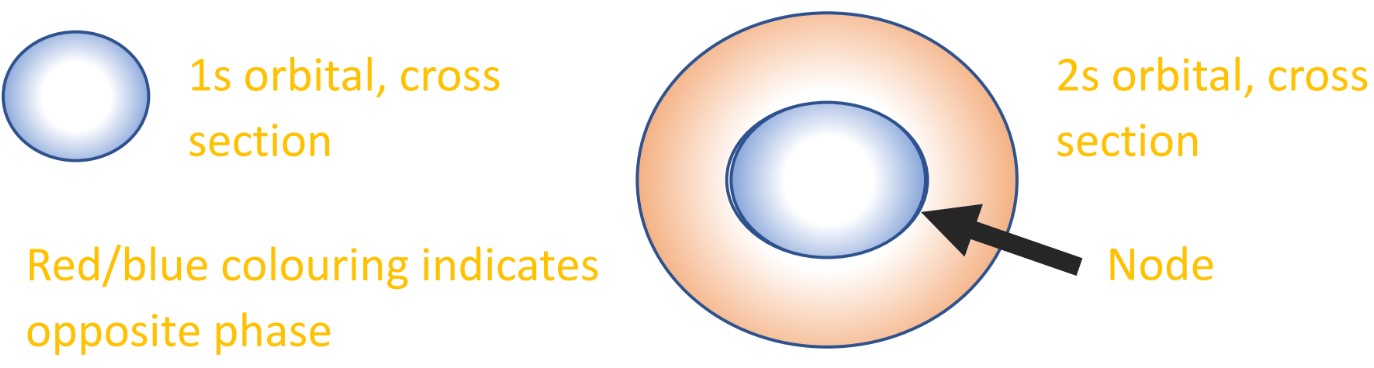
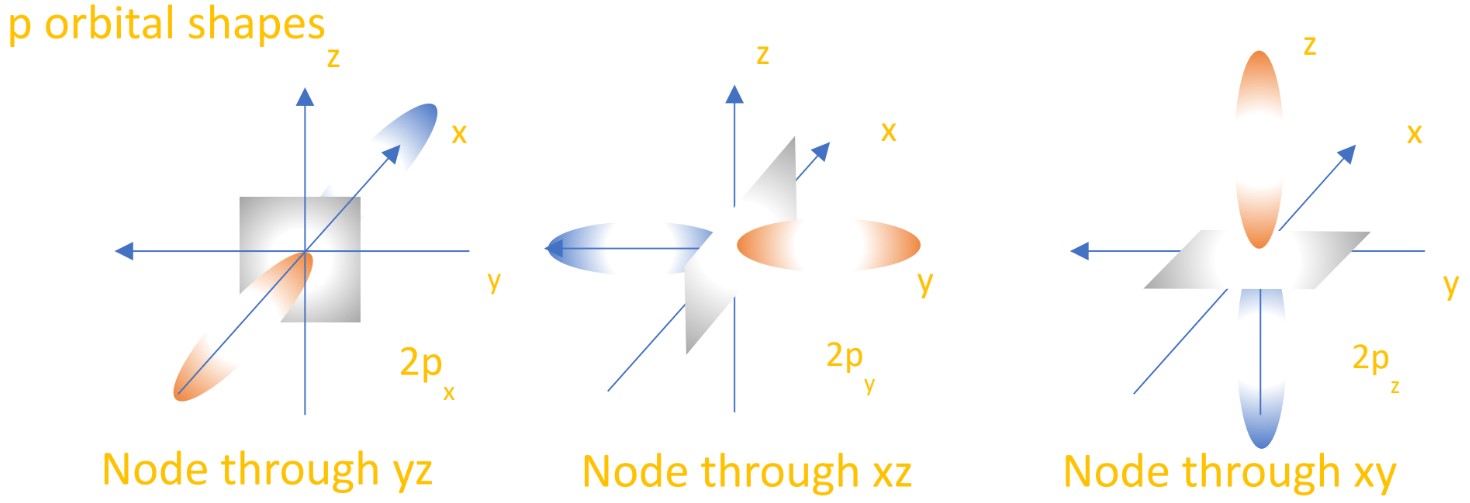
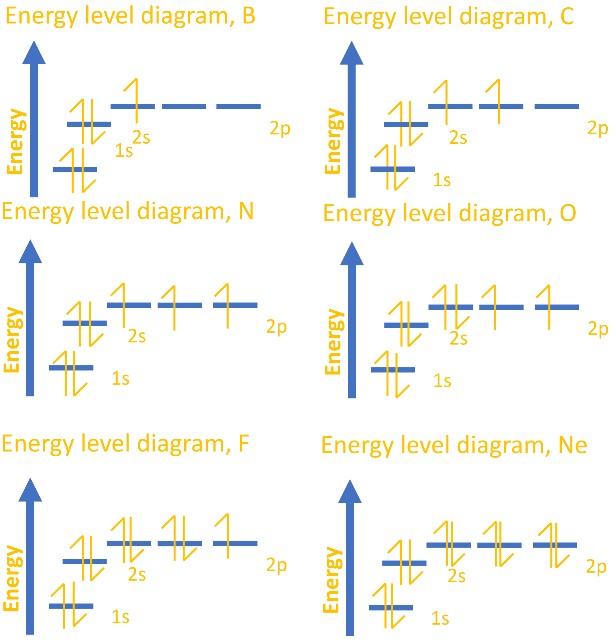